3.7: The Metric (Part 2)
( \newcommand{\kernel}{\mathrm{null}\,}\)
Isometry, Inner Products, and the Erlangen Program
In Euclidean geometry, the dot product of vectors a and b is given by
gxxaxbx+gyyayby+gzzazbz=axbx+ayby+azbz,
and in the special case where a = b we have the squared magnitude. In the tensor notation,
aμbν=a1b1+a2b2+a3b3.
Like magnitudes, dot products are invariant under rotations. This is because knowing the dot product of vectors a and b entails knowing the value of
a⋅b=|a||a|cosθab
and Euclid’s E4 (equality of right angles) implies that the angle θab is invariant. The same axioms also entail invariance of dot products under translation; Euclid waits only until the second proposition of the Elements to prove that line segments can be copied from one location to another. This seeming triviality is actually false as a description of physical space, because it amounts to a statement that space has the same properties everywhere.
The set of all transformations that can be built out of successive translations, rotations, and reflections is called the group of isometries. It can also be defined as the group that preserves dot products, or the group that preserves congruence of triangles.
GRoups
In mathematics, a group is defined as a binary operation that has an identity, inverses, and associativity. For example, addition of integers is a group. In the present context, the members of the group are not numbers but the transformations applied to the Euclidean plane. The group operation on transformations T1 and T2 consists of finding the transformation that results from doing one and then the other, i.e., composition of functions.
In Lorentzian geometry, we usually avoid the Euclidean term dot product and refer to the corresponding operation by the more general term inner product. In a specific coordinate system we have
aμbν=a0b0−a1b1−a2b2−a3b3.
The inner product is invariant under Lorentz boosts, and also under the Euclidean isometries. The group found by making all possible combinations of continuous transformations from these two sets is called the Poincaré group. The Poincaré group is not the symmetry group of all of spacetime, since curved spacetime has different properties in different locations. The equivalence principle tells us, however, that space can be approximated locally as being flat, so the Poincaré group is locally valid, just as the Euclidean isometries are locally valid as a description of geometry on the Earth’s curved surface.
CPT Symmetry
The discontinuous transformations of spatial reflection and time reversal are not included in the definition of the Poincaré group, although they do preserve inner products. General relativity has symmetry under spatial reflection (called P for parity), time reversal (T), and charge inversion (C), but the standard model of particle physics is only invariant under the composition of all three, CPT, not under any of these symmetries individually.
Example 16: The triangle inequality
In Euclidean geometry, the triangle inequality |b + c| < |b| + |c| follows from
(|b|+|c|)2−(b+c)⋅(b+c)=2(|b||c|−b⋅c)≥0.
The reason this quantity always comes out positive is that for two vectors of fixed magnitude, the greatest dot product is always achieved in the case where they lie along the same direction.
In Lorentzian geometry, the situation is different. Let b and c be timelike vectors, so that they represent possible world-lines. Then the relation a = b + c suggests the existence of two observers who take two different paths from one event to another. A goes by a direct route while B takes a detour. The magnitude of each timelike vector represents the time elapsed on a clock carried by the observer moving along that vector. The triangle equality is now reversed, becoming |b + c| > |b| + |c|. The difference from the Euclidean case arises because inner products are no longer necessarily maximized if vectors are in the same direction. E.g., for two lightlike vectors, bicj vanishes entirely if b and c are parallel. For timelike vectors, parallelism actually minimizes the inner product rather than maximizing it.
Proof
Let b and c be parallel and timelike, and directed forward in time. Adopt a frame of reference in which every spatial component of each vector vanishes. This entails no loss of generality, since inner products are invariant under such a transformation. Since the time-ordering is also preserved under transformations in the Poincaré group, each is still directed forward in time, not backward. Now let b and c be pulled away from parallelism, like opening a pair of scissors in the x − t plane. This reduces btct, while causing bxcx to become negative. Both effects increase the inner product.
◻
In his 1872 inaugural address at the University of Erlangen, Felix Klein used the idea of groups of transformations to lay out a general classification scheme, known as the Erlangen program, for all the different types of geometry. Each geometry is described by the group of transformations, called the principal group, that preserves the truth of geometrical statements. Euclidean geometry’s principal group consists of the isometries combined with arbitrary changes of scale, since there is nothing in Euclid’s axioms that singles out a particular distance as a unit of measurement. In other words, the principal group consists of the transformations that preserve similarity, not just those that preserve congruence. Affine geometry’s principal group is the transformations that preserve parallelism; it includes shear transformations, and there is therefore no invariant notion of angular measure or congruence. Unlike Euclidean and affine geometry, elliptic geometry does not have scale invariance. This is because there is a particular unit of distance that has special status; as we saw in example 4, a being living in an elliptic plane can determine, by entirely intrinsic methods, a distance scale R, which we can interpret in the hemispherical model as the radius of the sphere. General relativity breaks this symmetry even more severely. Not only is there a scale associated with curvature, but the scale is different from one point in space to another.
Einstein's Carousel
Non-Euclidean Geometry Observed in the Rotating Frame
The following example was historically important, because Einstein used it to convince himself that general relativity should be described by non-Euclidean geometry.8 Its interpretation is also fairly subtle, and the early relativists had some trouble with it.
Note
The example is described in Einstein’s paper “The Foundation of the General Theory of Relativity.” An excerpt, which includes the example, is given in Appendix A.
Suppose that observer A is on a spinning carousel while observer B stands on the ground. B says that A is accelerating, but by the equivalence principle A can say that she is at rest in a gravitational field, while B is free-falling out from under her. B measures the radius and circumference of the carousel, and finds that their ratio is 2π. A carries out similar measurements, but when she puts her meter-stick in the azimuthal direction it becomes Lorentz-contracted by the factor γ=(1−ω2r2)−1/2, so she finds that the ratio is greater than 2π. In A’s coordinates, the spatial geometry is non-Euclidean, and the metric differs from the Euclidean one found in example 8.
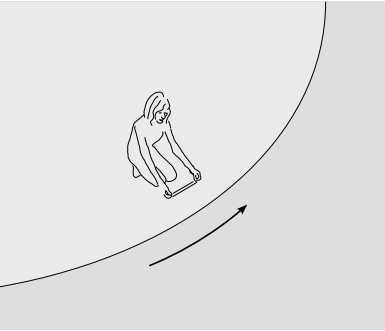
Observer A feels a force that B considers to be fictitious, but that, by the equivalence principle, A can say is a perfectly real gravitational force. According to A, an observer like B is free-falling away from the center of the disk under the influence of this gravitational field. A also observes that the spatial geometry of the carousel is non-Euclidean. Therefore it seems reasonable to conjecture that gravity can be described by non-Euclidean geometry, rather than as a physical force in the Newtonian sense.
At this point, you know as much about this example as Einstein did in 1912, when he began using it as the seed from which general relativity sprouted, collaborating with his old schoolmate, mathematician Marcel Grossmann, who knew about differential geometry. The remainder of this subsection, which you may want to skip on a first reading, goes into more detail on the interpretation and mathematical description of the rotating frame of reference. Even more detailed treatments are given by Grøn9 and Dieks.10
Ehrenfest’s Paradox
Ehrenfest11 described the following paradox. Suppose that observer B, in the lab frame, measures the radius of the disk to be r when the disk is at rest, and r' when the disk is spinning. B can also measure the corresponding circumferences C and C'. Because B is in an inertial frame, the spatial geometry does not appear non-Euclidean according to measurements carried out with his meter sticks, and therefore the Euclidean relations C = 2πr and C' = 2πr' both hold. The radial lines are perpendicular to their own motion, and they therefore have no length contraction, r = r', implying C = C'. The outer edge of the disk, however, is everywhere tangent to its own direction of motion, so it is Lorentz contracted, and therefore C' < C. The resolution of the paradox is that it rests on the incorrect assumption that a rigid disk can be made to rotate. If a perfectly rigid disk was initially not rotating, one would have to distort it in order to set it into rotation, because once it was rotating its outer edge would no longer have a length equal to 2π times its radius. Therefore if the disk is perfectly rigid, it can never be rotated. As discussed earlier, relativity does not allow the existence of infinitely rigid or infinitely strong materials. If it did, then one could violate causality. If a perfectly rigid disk existed, vibrations in the disk would propagate at infinite velocity, so tapping the disk with a hammer in one place would result in the transmission of information at v > c to other parts of the disk, and then there would exist frames of reference in which the information was received before it was transmitted. The same applies if the hammer tap is used to impart rotational motion to the disk.
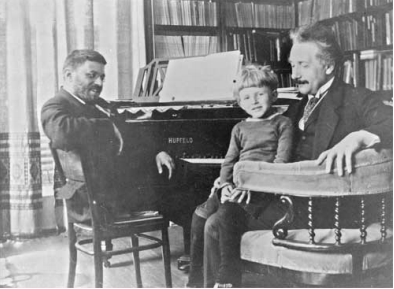
Exercise 3.7.1
Self-check: What if we build the disk by assembling the building materials so that they are already rotating properly before they are joined together?
The Metric in the Rotating Frame
What if we try to get around these problems by applying torque uniformly all over the disk, so that the rotation starts smoothly and simultaneously everywhere? We then run into issues identical to the ones raised by Bell’s spaceship paradox. In fact, Ehrenfest’s paradox is nothing more than Bell’s paradox wrapped around into a circle. The same question of time synchronization comes up.
To spell this out mathematically, let’s find the metric according to observer A by applying the change of coordinates θ′=θ−ωt. First we take the Euclidean metric of example 8 and rewrite it as a (globally) Lorentzian metric in spacetime for observer B,
ds2=dt2−dr2−r2dθ2.
Applying the transformation into A’s coordinates, we find
ds2=(1−ω2r2)dt2−dr2−r2dθ′2−2ωr2dθ′dt.
Recognizing ωr as the velocity of one frame relative to another, and (1−ω2r2)−1/2 as γ, we see that we do have a relativistic time dilation effect in the dt2 term. But the dr2 and dθ′2 terms look Euclidean. Why don’t we see any Lorentz contraction of the length scale in the azimuthal direction?
The answer is that coordinates in general relativity are arbitrary, and just because we can write down a certain set of coordinates, that doesn’t mean they have any special physical interpretation. The coordinates (t,r,θ′) do not correspond physically to the quantities that A would measure with clocks and meter-sticks. The tip-off is the dθ′ dt cross-term. Suppose that A sends two cars driving around the circumference of the carousel, one clockwise and one counterclockwise, from the same point. If (t, r, θ′) coordinates corresponded to clock and meter-stick measurements, then we would expect that when the cars met up again on the far side of the disk, their dashboards would show equal values of the arc length rθ′ on their odometers and equal proper times ds on their clocks. But this is not the case, because the sign of the dθ′ dt term is opposite for the two world-lines. The same effect occurs if we send beams of light in both directions around the disk, and this is the Sagnac effect.
This is a symptom of the fact that the coordinate t is not properly synchronized between different places on the disk. We already know that we should not expect to be able to find a universal time coordinate that will match up with every clock, regardless of the clock’s state of motion. Suppose we set ourselves a more modest goal. Can we find a universal time coordinate that will match up with every clock, provided that the clock is at rest relative to the rotating disk?
The Spatial Metric and Synchronization of Clocks
A trick for improving the situation is to eliminate the dθ′dt cross-term by completing the square in the metric (Equation ???). The result is
ds2=(1−ω2r2)[dt+ωr21−ω2r2dθ′]2−dr2−r21−ω2r2dθ′2.
The interpretation of the quantity in square brackets is as follows. Suppose that two observers situate themselves on the edge of the disk, separated by an infinitesimal angle dθ′. They then synchronize their clocks by exchanging light pulses. The time of flight, measured in the lab frame, for each light pulse is the solution of the equation ds2 = 0, and the only difference between the clockwise result dt1 and the counterclockwise one dt2 arises from the sign of dθ′. The quantity in square brackets is the same in both cases, so the amount by which the clocks must be adjusted is
dt=(dt2−dt1)2,
or
dt=ωr21−ω2r2dθ′.
Substituting this into the metric, we are left with the purely spatial metric
ds2=−dr2−r21−ω2r2dθ′2.
The factor of (1−ω2r2)−1=γ2 in the dθ′2 term is simply the expected Lorentz-contraction factor. In other words, the circumference is, as expected, greater than 2πr by a factor of γ.
Does the metric (Equation ???) represent the same non-Euclidean spatial geometry that A, rotating with the disk, would determine by meterstick measurements? Yes and no. It can be interpreted as the one that A would determine by radar measurements. That is, if A measures a round-trip travel time dt for a light signal between points separated by coordinate distances dr and dθ′, then A can say that the spatial separation is dt2, and such measurements will be described correctly by Equation ???. Physical meter-sticks, however, present some problems. Meter-sticks rotating with the disk are subject to Coriolis and centrifugal forces, and this problem can’t be avoided simply by making the meter-sticks infinitely rigid, because infinitely rigid objects are forbidden by relativity. In fact, these forces will inevitably be strong enough to destroy any meter stick that is brought out to r = 1ω, where the speed of the disk becomes equal to the speed of light.
It might appear that we could now define a global coordinate
T=t+ωr21−ω2r2θ′,
interpreted as a time coordinate that was synchronized in a consistent way for all points on the disk. The trouble with this interpretation becomes evident when we imagine driving a car around the circumference of the disk, at a speed slow enough so that there is negligible time dilation of the car’s dashboard clock relative to the clocks tied to the disk. Once the car gets back to its original position, θ′ has increased by 2pi, so it is no longer possible for the car’s clock to be synchronized with the clocks tied to the disk. We conclude that it is not possible to synchronize clocks in a rotating frame of reference; if we try to do it, we will inevitably have to have a discontinuity somewhere. This problem is present even locally, as demonstrated by the possibility of measuring the Sagnac effect with apparatus that is small compared to the disk. The only reason we were able to get away with time synchronization in order to establish the metric in Equation ??? is that all the physical manifestations of the impossibility of synchronization, e.g., the Sagnac effect, are proportional to the area of the region in which synchronization is attempted. Since we were only synchronizing two nearby points, the area enclosed by the light rays was zero.
Example 17: GPS
As a practical example, the GPS system is designed mainly to allow people to find their positions relative to the rotating surface of the earth (although it can also be used by space vehicles). That is, they are interested in their (r, θ′,ϕ) coordinates. The frame of reference defined by these coordinates is referred to as ECEF, for Earth-Centered, Earth-Fixed.
The system requires synchronization of the atomic clocks carried aboard the satellites, and this synchronization also needs to be extended to the (less accurate) clocks built into the receiver units. It is impossible to carry out such a synchronization globally in the rotating frame in order to create coordinates (T, r, θ′,ϕ). If we tried, it would result in discontinuities (see problem 8).
Instead, the GPS system handles clock synchronization in coordinates (t, r, θ′,ϕ), as in Equation ???. These are known as the Earth-Centered Inertial (ECI) coordinates. The t coordinate in this system is not the one that users at neighboring points on the earth’s surface would establish if they carried out clock synchronization using electromagnetic signals. It is simply the time coordinate of the nonrotating frame of reference tied to the earth’s center. Conceptually, we can imagine this time coordinate as one that is established by sending out an electromagnetic “tick-tock” signal from the earth’s center, with each satellite correcting the phase of the signal based on the propagation time inferred from its own r. In reality, this is accomplished by communication with a master control station in Colorado Springs, which communicates with the satellites via relays at Kwajalein, Ascension Island, Diego Garcia, and Cape Canaveral.
Example 18: Einstein’s goof, in the rotating frame
Example 10 recounted Einstein’s famous mistake in predicting that a clock at the pole would experience a time dilation relative to a clock at the equator, and the empirical test of this fact by Alley et al. using atomic clocks. The perfect cancellation of gravitational and kinematic time dilations might seem fortuitous, but it fact it isn’t. When we transform into the frame rotating along with the earth, there is no longer any kinematic effect at all, because neither clock is moving. In this frame, the surface of the earth’s oceans is an equipotential, so the gravitational time dilation vanishes as well, assuming both clocks are at sea level. In the transformation to the rotating frame, the metric picks up a dθ′ dt term, but since both clocks are fixed to the earth’s surface, they have dθ′ = 0, and there is no Sagnac effect.
Impossibility of Rigid Rotation, even with External Forces
The determination of the spatial metric with rulers at rest relative to the disk is appealing because of its conceptual simplicity compared to complicated procedures involving radar, and this was presumably why Einstein presented the concept using ruler measurements in his 1916 paper laying out the general theory of relativity.12 In an effort to recover this simplicity, we could propose using external forces to compensate for the centrifugal and Coriolis forces to which the rulers would be subjected, causing them to stay straight and maintain their correct lengths. Something of this kind is carried out with the large mirrors of some telescopes, which have active systems that compensate for gravitational deflections and other effects. The first issue to worry about is that one would need some way to monitor a ruler’s length and straightness. The monitoring system would presumably be based on measurements with beams of light, in which case the physical rulers themselves would become superfluous.
Note
The paper is reproduced in the back of the book, and the relevant part is in Appendix A.
In addition, we would need to be able to manipulate the rulers in order to place them where we wanted them, and these manipulations would include angular accelerations. If such a thing was possible, then it would also amount to a loophole in the resolution of the Ehrenfest paradox. Could Ehrenfest’s rotating disk be accelerated and decelerated with help from external forces, which would keep it from contorting into a potato chip? The problem we run into with such a strategy is one of clock synchronization. When it was time to impart an angular acceleration to the disk, all of the control systems would have to be activated simultaneously. But we have already seen that global clock synchronization cannot be realized for an object with finite area, and therefore there is a logical contradiction in this proposal. This makes it impossible to apply rigid angular acceleration to the disk, but not necessarily the rulers, which could in theory be one-dimensional.
References
9 Relativistic description of a rotating disk, Am. J. Phys. 43 (1975) 869
10 Space, Time, and Coordinates in a Rotating World, www.phys.uu.nl/igg/dieks
11 P. Ehrenfest, Gleichf¨ormige Rotation starrer K¨orper und Relativitätstheorie, Z. Phys. 10 (1909) 918, available in English translation at en.wikisource.org.