5.1: Measuring the Ages of Objects - Radiometric Dating
( \newcommand{\kernel}{\mathrm{null}\,}\)
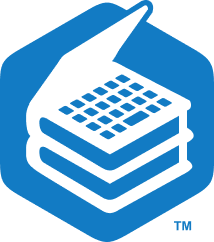
Login with LibreOne to view this question
NOTE: If you typically access ADAPT assignments through an LMS like Canvas, you should open this page there.
There are a variety of independent methods for measuring ages of different objects in the Universe, and these ages provide additional data to incorporate into our models of the Universe. Hopefully, this will give you an understanding of how we know the ages of various objects, so that they do not feel like random facts imposed from authority.
Radioactivity
At the turn of the 19th century, research into the phenomenon of radioactivity not only helped to radically transform our understanding of matter, but it allowed us to determine accurate ages for many materials for the first time.
Radioactivity is a process by which one atom undergoes a change in its nucleus, becoming a different kind of atom as it does so. These changes occur spontaneously, though similar changes can be artificially induced under the right conditions. The processes by which one type of atom spontaneously turns into another type are called nuclear decays.
Radioactivity was first reported in 1896 by Henri Becquerel (1852–1908), who noticed that samples containing uranium salts would expose photographic film, even if the film remained closed away in a box. In 1899, Ernest Rutherford (1871–1937) showed that the activity comes in two types, which he called alpha radiation and beta radiation. They were distinguished by their ability to penetrate through barriers. The alpha-type rays were more easily stopped than rays of the beta-type. A third type of radioactivity, termed gamma radiation, was discovered in 1900 by Paul Villard (1860–1934). A year later, Rutherford and his colleague, Frederick Soddy (1877–1956), showed that these radiation- producing processes are accompanied by the conversion of one type of atom into another type. Also in 1900, Becquerel showed that the beta rays emitted in the beta process are actually electrons. It took Rutherford and Thomas Royds (1884–1955) another six years to discover what the alpha particles are. They are discussed below.
The phenomenon of radioactivity was most thoroughly studied by the Polish/French chemist and physicist Marie Curie (1867–1934). Curie coined the term "radioactive" to describe these nuclei - a term that is unfortunate in that none of the so-called radioactive processes is associated with the emission of radio waves. Curie discovered radioactivity in thorium, and also discovered, along with her husband Pierre Curie (1859–1906), two new radioactive elements, polonium and radium.
It turns out that most naturally occurring atoms are stable; they do not undergo spontaneous decays. Only a small number of atoms are radioactive; this is because, over time, the unstable nuclei decay into stable ones. Nonetheless, radioactive atoms have played a large part in furthering our understanding of the atomic nucleus.
All chemical elements come in several different forms, known as isotopes. These have nuclei with slightly different masses due to a different number of neutrons. For instance, hydrogen, the simplest element, has three isotopes. The first, written 1H, has a nucleus containing just a single proton. The nucleus of the second form of hydrogen (2H) contains one proton and one neutron. This form of hydrogen is called deuterium. Finally, the third form of hydrogen (3H) is called tritium. Its nucleus contains a proton and two neutrons. Each form is denoted by the chemical symbol for hydrogen, with a superscript indicating the total number of protons plus neutrons. What makes each of these isotopes hydrogen is the single proton. Coupled with an electron it forms the hydrogen atom, as distinguished from the hydrogen nucleus. It is the electron that gives hydrogen its chemical properties.
Since it is the electrons that determine the chemical properties of atoms, each atomic isotope is chemically indistinguishable. For example, each isotope of hydrogen has a single electron and forms the same chemical compounds and undergoes the same chemical reactions. However, they do behave slightly differently in some respects. First, because they have different masses: Deuterium has twice the mass of 1H and tritium has three times the mass. So, as an example, water with deuterium is slightly heavier than water with regular hydrogen. Deuterium's extra weight makes it somewhat less likely to convert into vapor when water boils or evaporates.
Despite the chemical similarities of different isotopes of hydrogen, they behave very differently in nuclear reactions. This is especially true of tritium, which is radioactive. If we wait around for about 12 years, a tritium nucleus has about a 50% chance of turning into a 3He nucleus. This is a helium nucleus with two protons and one neutron. It is not guaranteed to do so, but there are even odds. If it does not decay in the first 12 years, then it has a 50% chance to decay in the next 12, and so on. In this decay, we would call tritium the parent nucleus and 3He would be called the daughter nucleus. Both normal hydrogen nuclei (protons) and deuterium nuclei are stable. They will not decay no matter how long we wait (at least, as far as we know), so tritium nuclei are strikingly different in this respect.
Each isotope of hydrogen has a single proton (that is what makes an isotope hydrogen), but each has a different numbers of neutrons. Similarly, helium has several isotopes. The most common has two neutrons to go along with its two protons and is called 4He. It is the alpha particle of alpha decay, as originally shown by Rutherford and Royds in 1906. Tritium decays into the other stable form of helium, 3He. There are four other isotopes of helium, but they are all unstable—in other words, radioactive.
In addition to hydrogen and helium, there are 90 additional kinds of atoms that occur naturally. They are listed in the Periodic Table of the Elements. Each one differs in the number of protons in its nucleus, and thus also in the number of electrons orbiting it nucleus. All of these atomic species have multiple isotopes, the nuclei of which differ only in their numbers of neutrons. Generally, most of the isotopes are unstable, but many atoms have more than one stable isotope. Some atomic species, the heaviest ones, have no stable isotopes at all.
It is not possible to predict exactly when a particular unstable nucleus will undergo a nuclear decay. This makes radioactivity a strictly non-Classical process; it can only be understood under the framework of quantum mechanics, just as is true for the distinct patterns of spectral lines emitted by atoms. We can know the probability that a nucleus will undergo a decay in a certain amount of time (for example, tritium has a 50% chance to decay in 12 years), but we cannot say exactly when that decay will occur for an individual nucleus.
The amount of time for half of a sample to decay is known as the isotope’s half-life. Again, since radioactive decay is a statistical process, it is not possible to predict exactly which atoms in the sample will decay and when, but we do know that approximately half of the sample will be gone in one half-life. Half of the remainder will decay after another half-life passes, and so on. The greater the number of atoms (N) in the sample, the better our accuracy, because the uncertainty in the number N equals the square root of N. For example, for a sample where we expected 100 atoms to decay after one half-life, the number actually decaying will typically be 100 ± √100 = 100 ± 10, or anywhere between 90 and 110. The percent uncertainty would be √N/N = 10/100 = 10%. In a sample with 1000 atoms, the uncertainty will be √1000 = 32, and we expect the number of decays to be roughly between 968 and 1032. This corresponds to uncertainty of 32/1000, or about 3%. In real samples used in the lab, typically the number of atoms is much, much greater than 100 (or 1000!), and so the percent uncertainty is correspondingly much lower.
If we have a sample of radioactive atoms in a lab, and if we measure the number of decay products it emits in a period of time, we will notice that it slowly drops the longer we watch the sample. And even though it is not possible to predict when any particular atom will decay, we can predict quite accurately how the number of decays in a large number of atoms will decrease over time.
As an example, imagine we had sample of 131I, a form of iodine used to treat certain types of cancers. If we measure the decay rate (by the detection of decay products, beta rays in this case), we would notice that after about 8 days the number of decays would have decreased by half. We could infer that our original sample would have only half of the original number of radioactive atoms of 131I. So if we started with 1000 131I atoms, after 8 days only 500 would be left. The rest of the iodine would have been converted to an isotope of the element xenon, 131Xe. After another 8 days, half the remaining iodine would have converted in turn, leaving only 250 in the sample. The other 250 would again have converted to xenon, and this pattern would repeat every eight days - see below for further discussion. In this example, iodine is called the parent nucleus, and xenon is the daughter.
In the decay process described above, the iodine nucleus starts out with 53 protons and 65 neutrons. In the decay process, one of the neutrons converts to a proton, an electron, and an antineutrino. The latter two are ejected from the nucleus, which is then left with 54 protons and 64 neutrons. The process is an example of beta decay because the radiation emitted includes an electron. In some nuclei, positrons are emitted instead of electrons, and neutrinos instead of antineutrinos. Positrons are the antimatter particle for electrons. They are identical to electrons but with positive electric charge rather than negative. Neutrinos and antineutrinos are also a matter/antimatter pair. Either way, the process is called beta decay (beta-plus or beta-minus, depending on whether a positron or electron is emitted).
A shorthand way of describing the decay of iodine above is to write it as an equation. These are similar to the chemical equations you might have learned in chemistry class, except here we have a nuclear reaction instead of a chemical reaction.
I131⟶Xe131+e−+ˉνe−
Equation ??? says that in the decay of iodine with atomic mass 131, the nucleus converts to xenon of atomic mass 131. In addition, an electron (e-) and an electron antineutrino ˉνe− (the bar over the neutrino symbol indicates it is an antineutrino) are emitted. Of course, what is really happening is that a single neutron in the nucleus of the iodine is converting to a proton. Since the proton and neutron have essentially the same atomic mass, and the electron and neutrino are almost massless by comparison, the atomic mass of the entire nucleus remains nearly constant in this process, with the daughter being only slightly lighter than the parent. We could write down the decay of a neutron (n) into a proton (p) in a manner similar to the equation above. This is the process occurring in any nucleus that decays by beta decay:
n⟶p++e−+ˉνe−
Xenon-131 is a stable nucleus, so it remains in our sample unchanged. As discussed above, if we continue for another 8 days, we will notice that the activity has been reduced further by half, to only one quarter of its original amount. Our sample will then contain only 250 atoms of iodine. The remaining 750 atoms will be xenon. In the next 8 days, the activity will reduce by half again. We will have only 125 atoms of iodine, and the remaining 875 atoms will be xenon. This continues, with half of the 131I converting to 131Xe every 8 days, until all the radioactive iodine has been converted to stable xenon. The total number of 131I and 131Xe nuclei (their sum) is constant. This is illustrated graphically in Figure 5.1.

In this activity, you will observe particles that undergo either alpha or beta decay, and you will have to determine which type of decay it is. Make sure you do enough examples to be comfortable identifying both alpha and beta decay.
When you begin the activity, you will see a linear table of elements, with a label on the highlighted element. The legend indicates that protons are represented by red particles, neutrons are gray, and electrons are smaller blue particles. Neutrinos are not shown.
- Click on the “next decay” button at the top of the application and a random element will be selected. Watch closely as the particle will soon decay into another element, and you will need to decide if an alpha or beta decay occurred. In the upper right-hand corner of the activity, it will display the original element’s name and the name of the element into which it decayed.
- Once you have decided on the type of decay you observed, click on your choice between the two buttons on the left, “alpha decay” or “beta decay.”
- Click “next decay” again to view another element’s decay.
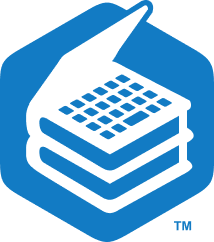
Login with LibreOne to view this question
NOTE: If you typically access ADAPT assignments through an LMS like Canvas, you should open this page there.
Determining Ages
This property of radioactive atoms makes them extremely useful as clocks. If we have a sample of material that contains radioactive elements, then we can use the relative amounts of the parent and daughter nuclei to deduce the number of half-lives that have passed since the sample was formed. Then, since we typically know the half-life in years, we can compute the age of the sample in years. This is how radiometric dating is done.
You may have heard of carbon dating, a method that uses the ratio of 14C to 12C to date organic samples, or items that were once-living things. For example, wood, charcoal, bones and shells can all be dated using the method of carbon dating. The ratio of 14C/12C (by number of nuclei) in CO2 molecules in Earth’s atmosphere is 1.3 × 10-12, and all living things have this same ratio because they constantly exchange CO2 with their surroundings. When an organism dies, this constant exchange ceases, and the 14C/12C ratio begins to decrease due to decay of 14C. Since the half-life of 14C is 5,730 years, it is a useful element to use to date objects that are between 1,000 and 25,000 years old. We use other radioactive elements to date older or younger objects or objects, such as rocks, that are not made of organic material.
In real samples, the process is somewhat more complicated than we have described. Real samples can be disturbed. Parts can be broken off, for example, or some atoms might evaporate or dissolve away. Or some amount of the daughter or parent species might be introduced by an outside agent. Furthermore, it is likely that some amount of the daughter nuclei is present in the material when it forms. The presence of this extra material can contribute to a spurious result for the age if it depends on just a single isotope.
In practice, a substance might contain several different kinds of radioactive elements, since essentially all atoms have some isotopes that are radioactive. It might also contain different minerals, each with different chemical properties. Each of these atoms or minerals can be analyzed separately, and an age computed from each of them. These ages will generally be close to each other, but not precisely the same. So some weighting of the individual ages based on their known chemical properties and the environment in which the sample was found can be used to determine an estimate of the true age, or to give a plausible range of ages. Uncertainties can be less than a percent or so (based upon the spread in ages from different parts of the sample). In certain instances they can be larger as well.
Some examples of common parent-daughter species are shown in the table below. The table also lists half-lives and typical ages that can be determined for each species. Note that the uranium decay chains, which are used in tandem to determine ages, are complex and involve intermediate unstable nuclei with much shorter half-lives than the primary one shown.
PARENT | DAUGHTER | HALF-LIFE (YR) | PROCESS | INTERMEDIATES | AGE (YR) |
---|---|---|---|---|---|
Uranium (235U) | Lead (207Pb) | 700 million | Alpha and beta | Th-Pa-Ac-Fr-Ra-At-Rn-Bi-Tl-Po | Millions to billions |
Uranium (238U) | Lead (206Pb) | 4.5 billion | Alpha and beta | Th-Pa-Ac-Fr-Ra-At-Rn-Bi-Tl-Po | Millions to billions |
Potassium (40K) | Argon (40Ar) | 1.3 billion | Beta plus (electron capture) | - | Thousands to billions |
Carbon (14C) | Nitrogen (14N) | 5,730 | Beta minus | - | Thousands |
Radiometric dating has been used to find absolute ages for rocks on Earth’s surface. For instance, many of Earth’s oldest rocks have been found in Northeastern Canada. They have ages of around 3.8 to 4.3 billion years. Radiometric dating has been used to assign ages to other rock layers in the geologic record, as shown in Figure 5.2. Rock samples from the surface of the Moon have also undergone radiometric analysis, giving ages for the lunar highlands (the light parts of the Moon) of about 4.4 billion years. The lunar maria (the dark areas) are younger, with ages of about 3.8 billion years. In addition, some of the oldest meteorite fragments have also been analyzed, with their ages being about 4.6 billion years. All of these ages point to the origin of the Solar System being slightly more than 4.5 billion years ago.

We can also place limits on the duration of the Solar System’s formation using radiometric methods. Primitive meteorites contain inordinate amounts of the isotope 26Mg; the more common form of magnesium, about 70% of the total, is 24Mg. 26Mg is formed from the decay of 26Al via beta-plus decay. 26Al has a half-life of only about 700,000 years, so the meteorites containing 26Mg must have formed, at most, a few million years after the formation of the 26Al, presumably in a supernova that predated the formation of the Solar System. This indicates that at least some of the building blocks of the Sun and planets were assembled fairly quickly after being synthesized in an earlier, now long-dead star.
In this activity, you are given a set of 400 red atoms. These atoms have a half-life of 5 seconds, and they decay into blue atoms.
1. Click the “start” button, and you will see the atoms decay over one half-life. At the end of this cycle, the counter will let you know the number of blue and red atoms that are now in the sample, as well as the percentage of red atoms remaining. There will still be a total of 400 atoms.
2. Predict: about how many red atoms will be present after another (second) half-life.
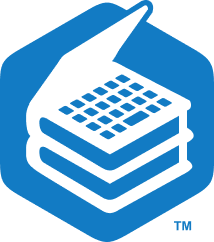
Login with LibreOne to view this question
NOTE: If you typically access ADAPT assignments through an LMS like Canvas, you should open this page there.
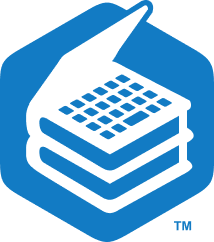
Login with LibreOne to view this question
NOTE: If you typically access ADAPT assignments through an LMS like Canvas, you should open this page there.
4. Predict: about how many blue atoms will be present after a third half-life is complete?
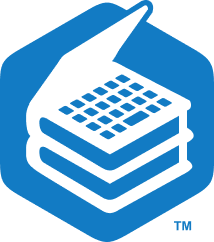
Login with LibreOne to view this question
NOTE: If you typically access ADAPT assignments through an LMS like Canvas, you should open this page there.
5. Click the “start” button to observe the third half-life. How did your predictions compare with your observations? Reconcile any discrepancies between your predictions and your observations and resolve them here.
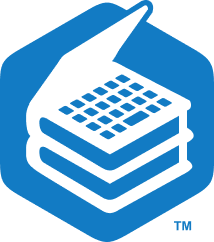
Login with LibreOne to view this question
NOTE: If you typically access ADAPT assignments through an LMS like Canvas, you should open this page there.
1. Can carbon dating be used to measure the age of a dinosaur? Explain.
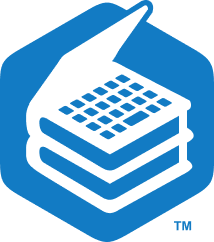
Login with LibreOne to view this question
NOTE: If you typically access ADAPT assignments through an LMS like Canvas, you should open this page there.
2. A 10.0 gram sample of 226Ra, which has a half-life of 1,660 years, is left to decay. How much of the original sample is left after one half-life?
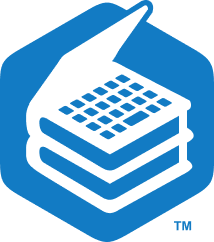
Login with LibreOne to view this question
NOTE: If you typically access ADAPT assignments through an LMS like Canvas, you should open this page there.
3. An archeologist finds some bones in an ancient burial site. The 14C content is 1/16 of that of living humans. How long ago did the ancient people live?
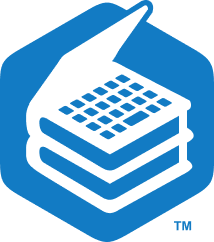
Login with LibreOne to view this question
NOTE: If you typically access ADAPT assignments through an LMS like Canvas, you should open this page there.
Explain your reasoning:
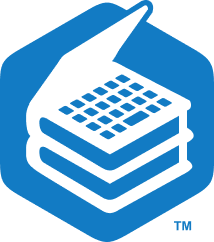
Login with LibreOne to view this question
NOTE: If you typically access ADAPT assignments through an LMS like Canvas, you should open this page there.
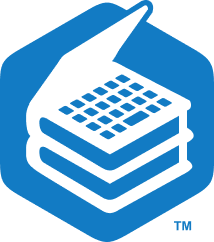
Login with LibreOne to view this question
NOTE: If you typically access ADAPT assignments through an LMS like Canvas, you should open this page there.
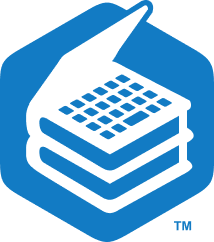
Login with LibreOne to view this question
NOTE: If you typically access ADAPT assignments through an LMS like Canvas, you should open this page there.
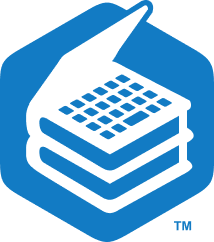
Login with LibreOne to view this question
NOTE: If you typically access ADAPT assignments through an LMS like Canvas, you should open this page there.