8.9: The First Law of Thermodynamics and Heat Engine Processes
( \newcommand{\kernel}{\mathrm{null}\,}\)
Learning Objectives
- Describe the processes of a simple heat engine.
- Explain the differences among the simple thermodynamic processes—isobaric, isochoric, isothermal, and adiabatic.
- Explain the relationship between work done by a gas and change in its volume.

One of the most important things we can do with heat transfer is to use it to do work for us. Such a device is called a heat engine. Car engines and steam turbines that generate electricity are examples of heat engines. Figure 8.9.2 shows schematically how the first law of thermodynamics applies to the typical heat engine.


The illustrations above show one of the ways in which heat transfer does work. Fuel combustion produces heat transfer to a gas in a cylinder, increasing the pressure of the gas and thereby the force it exerts on a movable piston. The gas does work on the outside world, as this force moves the piston through some distance. Heat transfer to the gas cylinder results in work being done. To repeat this process, the piston needs to be returned to its starting point. Heat transfer now occurs from the gas to the surroundings so that its pressure decreases, and a force is exerted by the surroundings to push the piston back through some distance. Variations of this process are employed daily in hundreds of millions of heat engines. Here, we consider some of the thermodynamic processes on which heat engines are based.
Work Done by a Gas
A process by which a gas does work on a piston at constant pressure is called an isobaric process. Since the pressure is constant, the force exerted is constant and the work done is given as
PΔV.

Recall from mechanics that work done by a force F on an object undergoing displacement d is
W=Fd.
See the symbols as shown in Figure 8.9.4. Now force is pressure times area (F=PA), and so
W= PAd .
Because the volume of a cylinder is its cross-sectional area A times its length d, we see that Ad=ΔV, the change in volume; thus,
W=PΔV (isobaric process) .
Note that if ΔV is positive, then W is positive, meaning that positive work is done by the gas on the outside world.
(Note that the pressure involved in this work that we’ve called P is the pressure of the gas inside the tank. If we call the pressure outside the tank Pext , an expanding gas would be working against the external pressure; the work done would therefore be W=−Pext ΔV (isobaric process). There are some—especially chemists—who use this definition of work, and not the definition based on internal pressure, as the basis of the First Law of Thermodynamics. This definition reverses the sign conventions for work, and results in a statement of the first law that becomes ΔU=Q+W. In this textbook, we will use the physics convention of using work done by the system on the surrounding, not the other way around.)
This is the key lesson from the above derivation: a gas expanding under pressure does work on its surrounding, and unless additional energy is added through heat transfer, the internal energy of the gas decreases. We will examine the experimental results that come about as a consequence of this fact later.
Thermodynamic Processes
We introduced the isobaric process above in discussing work done by a gas. Isobaric process is an example of a thermodynamic process. A thermodynamic process describes a change that happens to a gas, which results in change in its pressure (P), volume (V), and/or temperature (T). An isobaric process is a thermodynamic process that takes place under constant pressure (so the volume and temperature of the gas may change in an isobaric process).
There are three more named thermodynamic processes. These processes are given special names because, like the isobaric process, they occur under some restrictions, which gives them their special properties, as described briefly below. These three additional named thermodynamic processes are: isochoric, isothermal, and adiabatic processes.
An isochoric process is a thermodynamic process in which no change in volume takes place. Because the work done by a gas is proportional to the change in volume, in an isochoric process, no work is done by (or on) the gas. Instead, in an isochoric process, a heat transfer takes place, and the energy from the heat transfer goes into increasing (or decreasing) the internal energy of the gas, increasing (or decreasing) its temperature.
An isothermal process is a thermodynamic process in which no change in temperature takes place. A gas expanding isothermally, for example, does work on the surrounding, but its internal energy (as represented by the temperature) does not change, because enough heat flows in to balance out the energy expended in doing work. This is consistent with the first law of thermodynamics (0=ΔU=Q−W, because Q=W). An isothermal process occurs if a thermodynamic process in a gas occurs slowly enough so that the gas remains in thermal equilibrium with the surrounding at all times.
The adiabatic process is, in some sense, the opposite of an isothermal process. In an adiabatic process, no heat transfer takes place (that is, Q=0). This may happen because the gas is well-insulated from the surrounding. It may also happen because the process occurs so quickly that no significant heat transfer can take place. In an adiabatic expansion, for example, the internal energy of the gas decreases, because of the work done by the gas in expansion. This is perhaps the clearest experimental evidence one can observe that it takes work for a gas to expand under pressure.
Figure 8.9.5 illustrates these three processes on a plot of pressure and volume (a PV diagram). In an isothermal process, as the gas expands, the pressure decreases. This can be predicted from the ideal gas law (PV=NkT). Since the temperature is constant, if volume increases, the pressure must decrease, to keep PV constant. You can also see that in an adiabatic process, the pressure decreases with expanding volume more steeply than an isothermal process, because in an adiabatic process, the temperature is not constant, but it decreases. So with increasing V, the pressure decrease even more rapidly, so that PV actually decreases (for decreasing temperature).
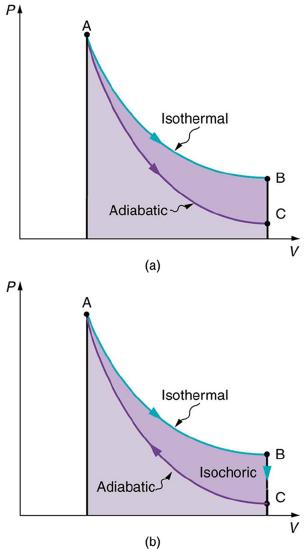
Reversible Processes
Both isothermal and adiabatic processes such as shown in Figure 8.9.5 are reversible in principle. A reversible process is one in which both the system and its environment can return to exactly the states they were in by following the reverse path. The reverse isothermal and adiabatic paths are BA and CA, respectively. Real macroscopic processes are never exactly reversible. In the previous examples, our system is a gas (like that in Figure 8.9.4), and its environment is the piston, cylinder, and the rest of the universe. If there are any energy-dissipating mechanisms, such as friction or turbulence, then heat transfer to the environment occurs for either direction of the piston. So, for example, if the path BA is followed and there is friction, then the gas will be returned to its original state but the environment will not—it will have been heated in both directions. Reversibility requires the direction of heat transfer to reverse for the reverse path. Since dissipative mechanisms cannot be completely eliminated, real processes cannot be reversible.
There must be reasons that real macroscopic processes cannot be reversible. We can imagine them going in reverse. For example, heat transfer occurs spontaneously from hot to cold and never spontaneously the reverse. Yet it would not violate the first law of thermodynamics for this to happen. In fact, all spontaneous processes, such as bubbles bursting, never go in reverse. There is a second thermodynamic law that forbids them from going in reverse. When we study this law, we will learn something about nature and also find that such a law limits the efficiency of heat engines. We will find that heat engines with the greatest possible theoretical efficiency would have to use reversible processes, and even they cannot convert all heat transfer into doing work. Table 8.9.1 summarizes the simpler thermodynamic processes and their definitions.
Isobaric | Constant pressure
W=PΔV |
---|---|
Isochoric | Constant volume
W=0 |
Isothermal | Constant temperature
Q=W |
Adiabatic | No heat transfer
Q=0 |
Section Summary
- One of the important implications of the first law of thermodynamics is that machines can be harnessed to do work that humans previously did by hand or by external energy supplies such as running water or the heat of the Sun. A machine that uses heat transfer to do work is known as a heat engine.
- There are several simple processes, used by heat engines, that flow from the first law of thermodynamics. Among them are the isobaric, isochoric, isothermal and adiabatic processes.
- These processes differ from one another based on how they affect pressure, volume, temperature, and heat transfer.
- If the work done is performed on the outside environment, work (W) will be a positive value. If the work done is done to the heat engine system, work (W) will be a negative value.
- Some thermodynamic processes, including isothermal and adiabatic processes, are reversible in theory; that is, both the thermodynamic system and the environment can be returned to their initial states. However, because of loss of energy owing to the second law of thermodynamics, complete reversibility does not work in practice.
Glossary
- first law of thermodynamics
- states that the change in internal energy of a system equals the net heat transfer into the system minus the net work done by the system
- internal energy
- the sum of the kinetic and potential energies of a system’s atoms and molecules
- human metabolism
- conversion of food into heat transfer, work, and stored fat